What you will learn in this 9-minute webinar:
- >> About the impact of increasing plastic use on the environment and human health.
- >> How Omni live-cell imaging can be used to monitor translocation of fluorescence-labeled microparticles in real time.
- >> How microparticle translocation across the intestinal epithelium appears to be size-dependent but not dose-dependent.
- >> How real-time kinetic monitoring on Omni minimizes the risk of potential interference caused by technical errors and/or leaching of fluorescence.
About the presenter:
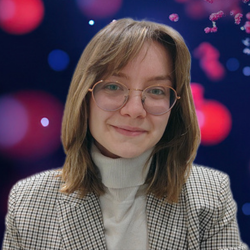
Maria Kloukinioti
Maastricht University
Maastricht, The Netherlands
Maria Kloukinioti completed her Master's degree at the University of Patras, Greece, in Management of Ecosystems and Biological Resources with a focus on ecotoxicology.
In 2022, she joined Maastricht University and TNO Institute as a PhD candidate in the field of Microplastics. Her research is centered on investigating the fate and effects of microplastics upon ingestion, using various in vitro and ex vivo intestinal models.
Transcript of the webinar:
Host: Thank you for joining our Coffee Break Webinar. Today's topic is "Tracking the translocation of microplastic particles."
Maria Kloukinioti completed her Master's degree at the University of Patras, Greece, in Management of Ecosystems and Biological Resources, with a focus on ecotoxicology. In 2022, she joined Maastricht University and the TNO Institute as a PhD candidate in the field of microplastics. Her research is centered on investigating the fate and effects of microplastics upon ingestion, using various in vitro and ex vivo intestinal models.
Maria Kloukinioti: Hello everyone, I'm really glad to be part of this Coffee Break Webinar series to present a part of my PhD research. The title of my presentation today is "Tracking the translocation of microplastic particles through live-cell imaging."
First, let me give you a bit of background on why plastic research is so important. As we all know and notice in our everyday lives, we use a vast amount of plastic products—from the clothes we wear to the packaging we use to store our food in.
Plastic use has grown exponentially over the years—and it doesn't seem to be slowing down. The problem with having so many plastic products around us is that, at the end of their lifespan and through constant use, these products begin to degrade and release smaller plastic particles called microplastics.
These particles are usually smaller than 5 mm and have a complex chemical composition. They also come in a variety of sizes and shapes. In general, we can divide microplastics into two major categories:
- Primary microplastics, which are particles intentionally produced and added to products like face creams and body scrubs. These particles are manufactured with a specific composition.
- Secondary microplastics, which result from the degradation of larger plastic products that have been released into the environment and exposed to various weathering processes.
Secondary microplastics have already been detected in different environments, including aquatic and terrestrial systems. They have also been found in our food chain. And if you're wondering which type poses a bigger risk, then we just have to look at the production numbers—and we can clearly see that secondary microplastics are far more abundant than primary ones.
A lot of research has already been conducted on microplastics, and it has been proven that these particles exist in human samples, such as blood and placenta. In our lab, in an effort to mimic the exposure of our gut to microplastics, we use an ex vivo system with porcine intestinal tissue. The tissue you see in the image on the screen is exposed to fluorescent polystyrene beads measuring 1 and 10 micrometers for six hours. After that, we attempt to track the uptake and translocation of the beads by these explants.
In this picture, you can clearly see that the microplastics are not just lying on the surface of the tissue—they are actively taken up by the cells as well. However, a major challenge remains: proving that these particles can actually cross the epithelium. The first issue is the small size of the microplastics and their limited rate of translocation. Existing methods, such as the use of a plate reader, which in theory can detect the fluorescent signal emitted by the microplastics, may not be sensitive enough to pick up the weak signal.
Additionally, in the field, there are growing concerns that fluorescence might leach out of the particles, resulting in false positive signals.
To overcome these challenges, we decided to use the Omni device from Axion BioSystems. This allows us to track translocated particles in real time, while also minimizing handling of the system—thereby reducing potential technical errors. Since our team had already demonstrated microplastic uptake by intestinal tissue (as I showed you earlier in the introduction), we decided to use the same model for studying translocation.
To explain a bit more about the setup of this model, let’s look at the image on the slide.
As you can see, we collect gut tissue and bring it to the lab, where we strip away the external muscle layer. We then punch the tissue into smaller round segments, which are mounted on a trans-well. In the end, we have a traditional trans-well setup you usually have for your cells, with an apical side, which is the exposure side, and a basolateral side, which is the receiving compartment for anything that might translocate—in our case, microplastics.
In this set of experiments, we used commercially available fluorescent polystyrene beads in three different sizes: 50 nanometers, 1 micrometer, and 10 micrometers. We also exposed our tissue segments to two different concentrations: 100,000 micrograms per milliliter, in order to explore whether size and dose could have an effect. The microscope was focused on the bottom of the tissue segment, as shown in the image below. This way, the moment a microplastic particle translocated, we could detect it.
In the beginning, we also took some test images to ensure that the microplastics were visible—both in a plain well plate and on the actual tissue segment. As you can see in the picture here, throughout all the videos we captured, we could clearly see 10 micrometer polystyrene beads passing through.
I’ll first play a video of one insert. Here, every small light that pops up on your screen represents one translocated particle. Of course, this isn’t very clear in real time, so next to the video, I’ve included screenshots of the microplastics you can see.
With the Omni device, we were able to track different locations on the same trans-well. At each time point, we could observe how many microplastics were passing through different regions of the same trans-well. What was particularly interesting was that we could detect some signal even with the 50-nanometer polystyrene exposures. Of course, I believe what we picked up were not single polystyrene beads, but rather aggregates, which are easier to detect. Still, it was exciting to see particles at this small scale.
Now, I understand that it might not be obvious from the video where exactly these particles appear. So, in the next slide, I’ve included a static image showing these particles. As I mentioned, in this image you can see red circles highlighting the translocated particles at the 50-nanometer size. They’re quite small, but we’re able to confirm their presence through these images, and not just background fluorescence.
From this small set of experiments, our preliminary conclusion is that it appears all three particle sizes were able to cross the intestinal epithelium. Translocation also seems to be size-dependent, as the 50-nanometer and 1-micrometer particles appeared earlier in our imaging than the 10-micrometer particles, whose translocation began a bit after the 3-hour mark. Of course, there may be some potential underestimation of translocation due to non-specific binding of particles to the support filter in the tissue segments. However, this is a common limitation when using trans-well setups.
For our next steps and future experiments, we aim to use more realistic particles—not just spherical ones, but also amorphous shapes. We also plan to test different polymer types to compare their translocation patterns and investigate whether there are notable differences.
To conclude, I’d really like to thank the entire team from TNO that helped me with this research, as well as the team at Maastricht University and everyone from the Momentum Consortium who made this collaboration possible and supported this research.